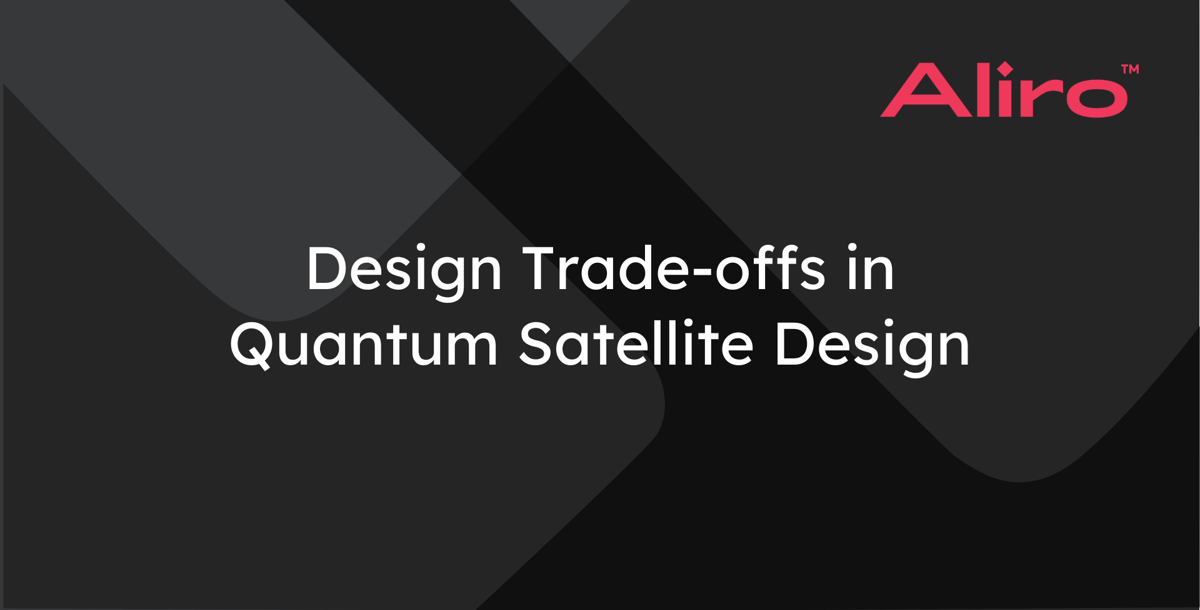
Designing a quantum satellite network requires careful evaluation of the factors impacting performance, scalability, and security. Design decisions involve trade-offs that must balance feasibility, efficiency, and long-term viability.
The key considerations in quantum satellite design, include:
- Satellite Orbit Selection. The selection of Low Earth Orbit (LEO), Medium Earth Orbit (MEO), or Geostationary Orbit (GEO) affects signal loss, transmission latency, and network coverage.
- QKD Protocol Selection. The selection of either a prepare-and-measure (BB84), an entanglement-based (Ekert-91, BBM92), or a measurement-device-independent (MDI-QKD) protocol affects security and complexity of network operations.
- Selecting Quantum Link Direction. The transmission of quantum signals, whether via uplink (ground-to-satellite) or downlink (satellite-to-ground), is affected differently by atmospheric signal distortion. This, in turn, influences the required distortion compensation scheme as well as impacts detection efficiency.
- Wavelength Selection and Photon Detectors. The chosen operational wavelength (e.g., C-band vs. Si-band) influences transmission loss, capability for daytime operation, detector sensitivity, and compatibility with existing quantum hardware.
Each of these design elements contribute to the efficiency and effectiveness of a quantum satellite network. In the following sections, we explore these trade-offs in detail, outlining their implications for global quantum communication and the future quantum internet.
Satellite Orbit Selection
The orbit of a satellite impacts quantum link performance, network scalability, and how the satellite operates. Each of the three main types of orbit offer advantages and challenges.
Image reference [23], [24]
The choice between LEO and MEO/GEO involves balancing key rate efficiency, coverage area, tracking complexity, and infrastructure costs. While LEO is preferred for high key-rate quantum links, MEO and GEO provide greater coverage. The challenges of these varying orbits are due to distance from the Earth, which leads to greater beam divergence, and from atmospheric effects that distort and tilt the beam. . Techniques such as adaptive optics and calibration techniques, such as using a beacon laser, can partially compensate for these effects.
QKD Protocol Selection
After choosing the optimal satellite orbit, the next design decision involves selecting the communication protocol. The selection of QKD protocol influences network security, operational complexity, and system performance
Image reference [10]
Prepare-and-measure protocols, such as BB84, utilize single qubits in superposition to distribute cryptographic keys. This approach offers several advantages, but there are security trade-offs to consider. Because these protocols require the satellite to be a trusted node (which may or may not be truly trustworthy), prepare-and-measure QKD protocols like BB84 introduce security vulnerabilities.
Entanglement-based protocols, such as BBM92, E91, and Measurement Device-Independent QKD (MDI-QKD), offer higher security by distributing entangled photon pairs between ground stations. That is, unlike prepare-and-measure protocols, entanglement-based QKD does not require trusting the satellite, making it more suitable for high-security applications.
Selecting Quantum Link Direction
The choice of quantum link direction, uplink (ground-to-satellite) or downlink (satellite-to-ground), also impacts the performance and feasibility of satellite-based quantum communication systems. There are two metrics that help to quantify the trade-offs between uplink and downlink configurations:
Point Ahead Angle (PAA). Point Ahead Angle refers to the angular difference between the direction a transmitter needs to point its beam and the direction of the intended receiver; essentially, it's the angle at which the transmitter needs to aim slightly ahead of the receiver's current position to ensure the signal reaches it properly. Misalignment of PAA reduces efficiency at the receiver.
Isoplanatic Angle. Isoplanatic angle refers to the separation of two optical beams in the sky that overlap enough that one of these beams can be used to correct the effects of atmospheric turbulence on the other beam.
Downlink Example
In a downlink configuration, where quantum signals are transmitted from the satellite to the ground station, the primary challenge is wavefront distortion. This distortion occurs due to turbulence in the lower 10 km of the atmosphere, where fluctuations in the refractive index alter the shape of the wavefront and introduce phase errors.
This distortion can be mitigated using adaptive optics, which correct the incoming wavefront in real time. Downlink configurations also experience minimal beam wandering compared to uplinks. Beam wandering refers to the lateral displacement of the beam's center due to large-scale atmospheric turbulence. In uplinks, the beam experiences tilt early in its propagation and travels a long distance afterward, resulting in a significant shift in beam center. In contrast, downlink beams encounter turbulence in the final segment of propagation, leading to less pronounced displacement.
Most existing and planned quantum satellite missions use downlink, and use established ground-based technologies for signal correction.
Uplink Example
In an uplink configuration, where the quantum signal is transmitted from a ground station to a satellite, beam wandering is the main issue that hinders a stable link with a moving satellite. The tilt experienced by the uplink beam while propagating through the atmosphere leads to a significant shift in the beam center as it continues traveling through free space, severely degrading signal collection efficiency. When the point-ahead angle is less than or comparable to the isoplanatic angle, beam wandering can be corrected using a downlink beacon, as the propagation paths of both beams sufficiently overlap. However, for low Earth orbits, this correction becomes more difficult because the high orbital speeds can cause the point-ahead angle to exceed the isoplanatic angle, making a downlink beacon an invalid reference for correction.
Wavefront distortion has a lesser impact on signal quality in an uplink configuration compared to a downlink configuration. This is because, in an uplink setup, most wavefront distortion occurs within the first 10 km of atmospheric propagation, before the beam undergoes significant divergence in free space. As the beam expands, the fast irregularities introduced by the atmosphere remain nearly unchanged and are further stretched out, reducing their degrading effect on the signal.
Choosing the Right Link Direction
Many quantum satellite missions have opted for downlink QKD, benefiting from adaptive optics correction and reduced beam wandering in this configuration. Upcoming quantum missions are planned to explore uplink configurations and further improve this configuration for quantum communications. As quantum satellite technology progresses, hybrid configurations that combine both uplink and downlink topologies for the quantum links could offer a more robust and resilient global quantum network.
Selecting Wavelength
The selection of wavelength for quantum satellite communication is a design choice that impacts transmission efficiency, hardware compatibility, detector performance, and resilience to atmospheric noise. The two common wavelength bands chosen for quantum satellite communication are the telecommunications band, or C-band (1530–1565 nm), and the silicon band, or Si-band (700 - 900 nm). As with the other design considerations, the selection of wavelength has benefits and trade-offs.
Image reference [10], [27], [28]
The C-band leverages existing fiber infrastructure and offers strong daytime performance, but typically requires cryogenic detectors that introduce size, weight, and power constraints. In contrast, Si-band detectors can operate at room temperature with more relaxed SWaP requirements, making them well-suited for spaceborne payloads. They also experience less beam divergence, supporting higher key rates. However, they are more susceptible to solar noise, making them unsuitable for daytime operation, and are not compatible with existing fiber optic infrastructure.
Selecting Photon Sources
The selection of photon sources is dependent on the QKD protocol being implemented, with different requirements for using prepare-and-measure protocols like BB84 versus using entanglement-based protocols like BBM92 and Ekert91 (E91). Practical considerations such as temperature stability, power fluctuations, and integration with satellite-to-ground and satellite-to-satellite links are important when selecting the best photon source for a quantum payload.
Image reference [34], [35], [36]
For prepare-and-measure QKD (BB84), the commonly used photon source is the weak coherent pulse. One advantage of using weak coherent sources is that they produce higher key rates. Single photon sources using quantum dots and other emitters are another photon source used in prepare-and-measure QKD. These sources have lower key rates, but are maturing for space-based deployment.
For entanglement-based QKD (BBM92 and EK91), entangled photon pairs are distributed between nodes, whether those nodes are ground stations or satellites. This requires the use of entangled photon sources, which are discussed in detail in the white paper Entangled Photon Sources. Many entangled photon sources are available for utility, but when placing them in a payload, there are constraints to consider such as temperature fluctuations and power constraints.
Conclusion
Designing a quantum satellite network requires balancing competing factors. The selection of orbit, QKD protocol, link direction, wavelength, and photon sources each determine network efficiency, security, and feasibility. As quantum satellite technology advances, the security and scalability of quantum communication networks will continue to grow.
References
[10] Orsucci, Davide, et al. "Assessment of practical satellite quantum key distribution architectures for current and near-future missions." arXiv preprint arXiv:2404.05668 (2024).
[23] Tsai, Wen-Shing, et al. "A 30 Gb/s PAM4 underwater wireless laser transmission system with optical beam reducer/expander." Scientific reports 9.1 (2019): 8605
[24]https://www.groundcontrol.com/knowledge/guides/satellite-orbit-heights-impact-satellite-communication/
[27] https://www.laserworld.com/en/divergence.html
[28] Liao, Sheng-Kai, et al. "Long-distance free-space quantum key distribution in daylight towards inter-satellite communication." Nature Photonics 11.8 (2017): 509-513.
[34] https://en.wikipedia.org/wiki/Spontaneous_parametric_down-conversion
[35]https://quantumconsortium.org/wp-content/uploads/2021/08/QED-C-Marketplace-Qunnect-N.Goddard-Entanglement-Sources.pdf
[36] https://www.quandela.com/technology/the-power-of-single-photon-sources/
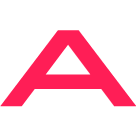