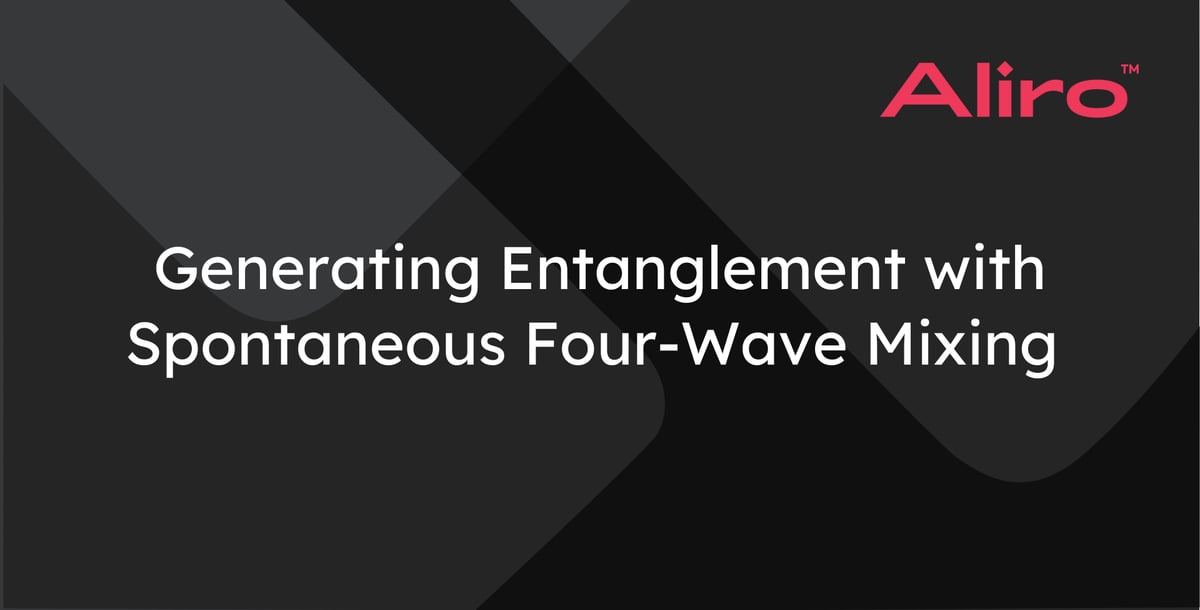
SPDC is a three-wave mixing process in which a single high-frequency pump photon produces a photon pair at lower frequencies through a nonlinear process mediated by the second-order nonlinear susceptibility, χ(2). Materials with a significant χ(2) susceptibility include bulk crystals such as beta-barium borate (BBO), potassium titanyl phosphate (KTP), and lithium niobate (LN).
Spontaneous Four-Wave Mixing (SFWM)
In contrast, SFWM is a third-order nonlinear process that arises in media with a significant χ(3) susceptibility.
In SFWM:
- Two pump photons from a strong input beam interact within the nonlinear medium.
- Through quantum-mechanical interactions, the medium generates two entangled photons: a signal photon and an idler photon.
The interaction Hamiltonian governing this process describes photon annihilation and creation. Simplified, the Hamiltonian shows that two pump photons are "annihilated" to simultaneously create a signal photon and an idler photon. This results in a quadratic dependence of the emitted flux on pump power, as opposed to the linear dependence seen in SPDC. Unlike SPDC, where the pump frequency is significantly higher than the generated photons, all photons involved in the SFWM process typically lie within a similar frequency band.
Amorphous materials like glass exhibit a significant χ(3) term. Furthermore, SFWM is feasible in silicon-based photonic platforms and other widely used materials like Silicon Nitride compatible with existing semiconductor fabrication technologies. While SPDC is a well-known method, SFWM is gaining popularity for generating entangled photons due to its compatibility with these platforms. Furthermore, these platforms allow for generation of entangled photons at telecom wavelengths (1550 nm) via SFWM, which are critical for compatibility with classical optical fiber networks.
Challenges, Applications, Efficiency and Phase matching
SPDC relies on the stronger second-order nonlinearity, χ(2), and typically achieves a higher pair generation rate than SFWM, which uses the weaker third-order nonlinearity, χ(3) . SPDC processes however often need to employ birefringent phase matching or quasi-phase matching schemes to overcome phase mismatches between the pump frequency and the generated signal and idler frequencies. For further details on these schemes, see our on-demand webinar Quantum Networking Hardware: Entangled Photon Sources.
In comparison, SFWM can generate photons within a similar frequency band to the pump light, simplifying the fulfillment of phase-matching requirements. For example, phase matching is often achieved by tailoring the dispersion properties of the medium/waveguide. Additionally, different spatial modes in multi- or few-mode waveguides with different effective indices can also be utilized to achieve phase matching.
A notable challenge in SFWM arises from spontaneous Raman scattering (SpRS) noise in optical fibers, which degrades the quality of the generated photon pairs. This noise is suppressed by filtering the pump after generation and can be further mitigated through cryogenic cooling—though the latter substantially increases implementation costs.
SPDC naturally supports the generation of polarization-entangled photon pairs (e.g., in Type-II SPDC). While SFWM does not inherently generate polarization-entangled photons, there have been novel designs that have allowed for this generation. SFWM photon-pair sources have been implemented across a variety of platforms like single and few mode fibers, photonic crystal fibers as well as integrated waveguides and ring resonators. This versatility makes designing SWFM process in waveguides and fibers highly adaptable, offering diverse source characteristics such as operation over single or multiple spatial modes, operation at different geometries such as counter- or co-propagating as well as cross- or co-polarized photons traversing the nonlinear medium. These diverse design capabilities have enabled the use of SFWM for demonstrations of entanglement in polarization, frequency, transverse mode, or time-bin degrees of freedom, as well as hyperentanglement across multiple degrees of freedom. Hyperentanglement occurs when photons exhibit entanglement across two or more degrees of freedom (e.g., polarization and frequency), expanding the available Hilbert space and enabling advanced quantum protocols by enhancing the capacity for dense coding, allowing for single-copy entanglement distillation, and many more.
Thus, Spontaneous Four-Wave Mixing is a powerful technique for generating entangled photons, with advantages in material versatility, on-chip integration via semiconductor fabrication technologies, and the ability to create on-chip hyperentanglement.
References
- Moreno, Jamy. Spontaneous four-wave mixing in standard birefringent fiber. Diss. University of Delaware, 2012.
- Garay-Palmett, Karina, et al. "Fiber-based photon-pair generation: tutorial." JOSA B 40.3 (2023): 469-490.
- Lee, Ju Han, Kyungtaek Lee, and Junha Jung. "Polarization-entangled photon pairs in the visible spectral band using intermodal spontaneous four-wave mixing." (2022).
- Wang, Haoyang, et al. "Progress on chip-based spontaneous four-wave mixing quantum light sources." Advanced Devices & Instrumentation 5 (2024): 0032.
- De la Torre-Robles, Daniel, et al. "Frequency and polarization emission properties of a photon-pair source based on a photonic crystal fiber." Scientific Reports 11.1 (2021): 18092.
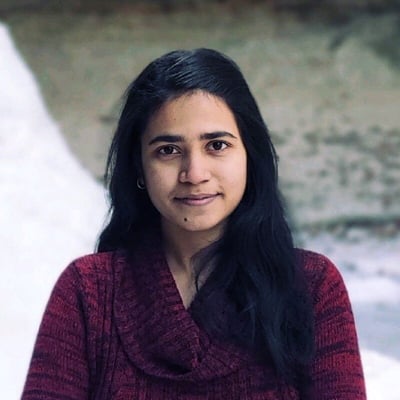