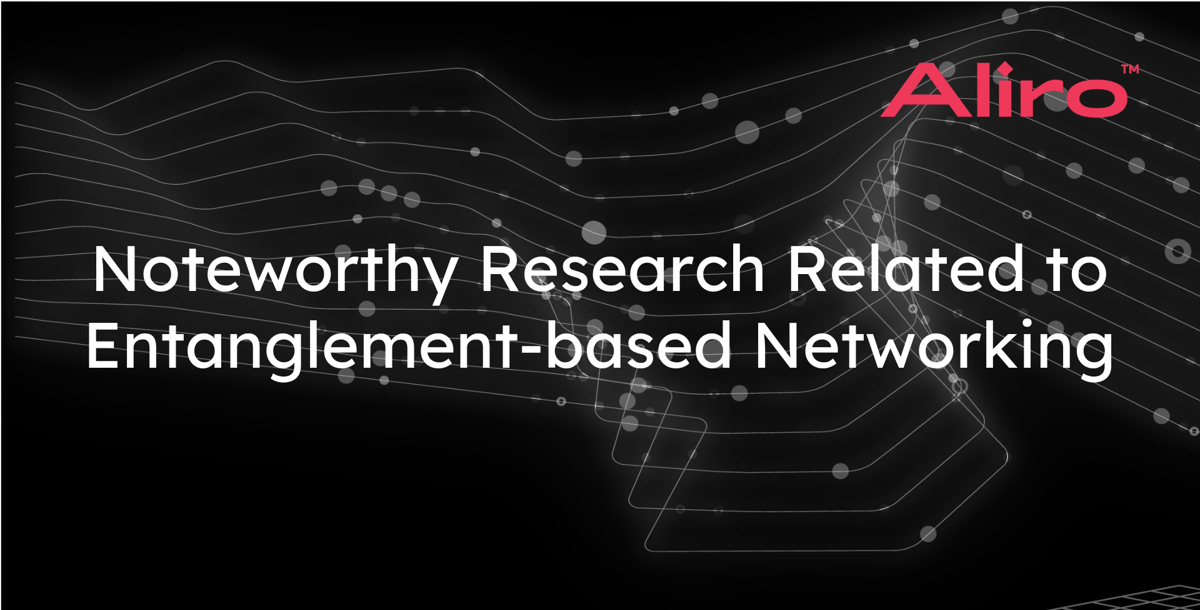
The rapid pace of breakthroughs in entanglement-based quantum networking demands attention, but it’s challenging to keep up with new research that is constantly occurring - and to understand the implications of that research. In this blog post, we aim to give a recap of recent and impactful research, straightforward explanations of breakthroughs pertaining to entanglement-based quantum networks, the implications of recent research related to quantum networks, and how this research is shaping the future of secure communication.
1. A long-distance quantum-capable internet testbedAuthored by: Dounan Du,1 Leonardo Castillo-Veneros,1 Dillion Cottrill,1 Guo-Dong Cui,1 Gabriel Bello,2 Mael Flament,2 Paul Stankus,3 Dimitrios Katramatos,3 Julia ́n Mart ́ınez-Rinco ́n,3 and Eden Figueroa1, 3
1 Department of Physics and Astronomy, Stony Brook University, Stony Brook, NY 11794, USA
2 Qunnect Inc., 141 Flushing Av. Suite 1110, Brooklyn, NY, 11205, USA
3 Brookhaven National Laboratory, Upton, NY, 11973, USA
https://arxiv.org/pdf/2101.12742.pdf
From the abstract: “Building a Quantum Internet requires the development of new networking concepts at the intersection of frontier communication systems and long-distance quantum communication. Here, we present the implementation of a quantum-enabled internet proto- type, where we have combined Software-Defined and Time-Sensitive Networking principles with Quantum Communication between quantum memories. Using a deployed quantum network connecting Stony Brook University and Brookhaven National Laboratory, we demonstrate a fundamental long-distance quantum network service, that of high-visibility Hong-Ou-Mandel Interference of telecom photons produced in two independent quantum memories separated by a distance of 158 km.”
This paper is bringing together industry, a national lab, and a university and showing a great advancement in long-distance quantum network implementation and control, and specifically in the areas of integrating some classical network concepts like a network stack. Researchers are producing two photons using two separate quantum memories, and they are sending these photons over a distance of 158 kilometers to be interfered via HOM interference and they're demonstrating this interference between these two photons.
This work is using room temperature quantum memories, specifically rubidium vapor cells. In this work, they're also using or implementing quantum frequency conversion between telecom and near-IR frequencies. The challenges that are addressed in this paper are:
- Implementation of a long distance quantum network. The distance that these these photons are traveling over is 158 kilometers.
- Creation of a quantum network stack and quantum network orchestrator, bringing in some existing classical networking concepts into the quantum networking world.
- Polarization compensation and time synchronization implementation.
The paper illustrates a quantum network stack schematic and shows three layers. First, there is a classical network layer. This layer has different components, including white rabbits, multiplexer, orchestrator, node controllers, network switches, and these are all sending like TCP/IP signals and sending classical signals for controlling this quantum network. Beneath this layer is the quantum enabling network layer, which contains polarization control and compensation, and preparation. This layer is bridging the interface between the classical network layer and the last layer: the quantum network layer. The quantum network layer contains the rubidium vapor cell memories and the photons, which are used for HOM interference. HOM interference is performed on the quantum layer, as is the quantum frequency transduction. In this particular network architecture, researchers are studying the quantum signals over one fiber and classical signals over another separate fiber.
Another advancement in this paper is their polarization compensation technique. In a figure showing polarization compensation data as a plot of fidelity with time, the plot shows that during nighttime hours, the fidelity is quite high with very little fluctuation. There aren't many perturbations to the quantum state that the photon is carrying. However, during the day, there are a lot of drops in fidelity. This is occurring because many kilometers of optical fiber for this network are laid over or along roads. During the day when people are out driving their cars, there are small changes in temperature and pressure that are perturbing the fibers. Even these tiny fluctuations are able to perturb the quantum state of the photon. However, every 15 minutes the fidelity rises back up to approximately one. This is due to the use of a very effective polarization compensator to correct for these drifts in polarization that can occur.
To sum up this paper overall: it's a great advance in a long distance quantum network implementation using room temperature quantum memories, and also incorporating polarization compensation and and of course, time synchronization and some classical networking concepts and tools as well.
2. Robust quantum memory in a trapped-ion quantum network node
Authored by: P. Drmota, D. Main, D. P. Nadlinger, B. C. Nichol, M. A. Weber, E. M. Ainley, A. Agrawal, R. Srinivas, G. Araneda, C. J. Ballance, D. M. Lucas
https://arxiv.org/abs/2210.11447
From the abstract: “We integrate a long-lived memory qubit into a mixed-species trapped-ion quantum network node. Ion-photon entanglement first generated with a network qubit in Sr-88 is transferred to Ca-43 with 0.977(7) fidelity, and mapped to a robust memory qubit. We then entangle the network qubit with a second photon, without affecting the memory qubit. We perform quantum state tomography to show that the fidelity of ion-photon entanglement decays ~70 times slower on the memory qubit. Dynamical decoupling further extends the storage duration; we measure an ion-photon entanglement fidelity of 0.81(4) after 10s.”
This is a paper from the University of Oxford, and researchers are using trapped ions as quantum memories for quantum networking. Some of the challenges this paper confronts are:
- Creation of a reliable and long lived memory and error correction.
- High fidelity quantum gates.
Strontium ions are used to produce ion-photon entanglement. This entanglement is then transferred from the strontium ion to a longer lived storage qubit: a calcium ion. Some noise reduction techniques are then applied to this calcium ion in order to extend the lifetime of the quantum memory and reduce noise.
Integration of different types of quantum memories, whether it be different types of ions or other platforms, will be crucial to building successful quantum networks. This paper is exploring this challenge head-on. Researchers entangled a photon with a strontium ion and are able to produce this entanglement at a fidelity as high as .97. However, the strontium ion has a relatively short coherence time. This initially high fidelity drops right away. When researchers swap entanglement from the photon strontium pair to the photon calcium pair, there is an initial hit to fidelity, down to .93, but the coherence time is increased quite a bit. There is magnetic field noise and noise from the ions being in the laser interaction region, which impacts the coherence time and fidelity for the quantum state. When ions are transferred away from the laser interaction region, and combined with implementing dynamical decoupling, researchers were able to see much longer coherence times - of up to 10 seconds - with fidelity of about 0.80.
Performing quantum gates and quantum logic on quantum memories is also crucial for second and third generation quantum networks, and the work in this paper highlights a path forward in this area. Researchers use an improved swap gate, which can swap the states while introducing fewer errors. In particular, they're able to do this swap with a process fidelity of only about 0.91, and with additional error correction they're able to improve that fidelity to 0.98.
To sum up this paper, this is excellent research showing how trapped ions can be used as quantum memories in quantum networks, and how noise reduction techniques can be used to extend their lifetimes. It also showcases the implementation of an iSWAP gate.
3. Architecture of a First-Generation Commercial Quantum Network
Authored by: Duncan Earl, K Karunaratne, Jason Schaake, Ryan Strum, Patrick Swingle, Ryan Wilson
Qubitekk, Inc., Vista, CA, 92081 USA
https://arxiv.org/pdf/2211.14871.pdf
From the abstract: “We present the architecture and near-term use cases for a first-generation commercial quantum network. We define the foundational hardware and software elements required to operate and manage the network. Finally, we discuss the configuration of this network for near-term consumer applications and propose how the network can support the broader technical goals of the quantum information science community.”
This is a paper from Qubitekk, so it comes entirely from the commercial sector. This paper is essentially introducing a commercial quantum network that can be used to accelerate the development of quantum devices, advance research, and is completely controllable and configurable by classical network administrators while also being scalable.
The challenges that are addressed with this paper are:
- Moving from the university setting to a commercial setting.
- The creation of a quantum network stack.
- Use of a quantum network orchestrator.
This quantum network is using the Aliro orchestrator and using existing classical networking tools like NETCONF and YANG to configure and monitor equipment, as well as polarization compensation and time synchronization and implementation of a scalable architecture.
This paper shows a schematic of the quantum network and existing components of it:
- Optical fibers. A total of 12, some carrying quantum signals and some carrying classical signals.
- Large fiber optical switches (20 by 20 and 60 by 60). These are quantum compatible optical switches: they have extremely low crosstalk.
- Prepare modules
- Measure modules
- A qubit source: a type two spontaneous parametric down conversion source at 1570 nanometers
- SNSPDs as detectors, which are really high efficiency detectors
- Serial ports and virtual machines, for classical control of some equipment.
Also in this paper is a diagram showing the progression of this quantum network. Implemented in this quantum network already is the bottom row of the diagram, which includes fiber optical switches, precision timing, control plane software and high efficiency detectors. The diagram builds up with more elements of the diagram put in place as this quantum network evolves. This will mean adding quantum memories, adding on-demand qubits versus frequency converters.
The main takeaway from this paper is that it’s really showing where entanglement-based Quantum Networking technology is heading through the lens of this first commercial quantum network, which is also integrating a lot of great existing classical networking tools.
authored by Guus Avis, Filip Rozpędek, Stephanie Wehner
https://arxiv.org/abs/2203.05517
From the abstract: “We study the performance (rate and fidelity) of distributing multipartite entangled states in a quantum network through the use of a central node. Specifically, we consider the scenario where the multipartite entangled state is first prepared locally at a central node, and then transmitted to the end nodes of the network through quantum teleportation. As our first result, we present leading-order analytical expressions and lower bounds for both the rate and fidelity at which a specific class of multipartite entangled states, namely Greenberger-Horne-Zeilinger (GHZ) states, are distributed. Our analytical expressions for the fidelity accurately account for time-dependent depolarizing noise encountered by individual quantum bits while stored in quantum memory, as verified using Monte Carlo simulations. As our second result, we compare the performance to the case where the central node is an entanglement switch and the GHZ state is created by the end nodes in a distributed fashion. Apart from these two results, we outline how the teleportation-based scheme could be physically implemented using trapped ions or nitrogen-vacancy centers in diamond.”
This paper explores ways of distributing multipartite entanglement that could be achieved within a network setting.
A typical bipartite entanglement is between two parties. For bipartite entanglement between just two parties, there is just one type of entanglement. These parties have a Bell state between them, which is a resource they can use for quantum communication. In bipartite entanglement, there are different Bell states, but they are all of the same type of state - they can be transformed into each other just by one of the parties performing an operation on their own. It doesn't require more entanglement to change one Bell state into another Bell state.
When more than two parties are entangled, the kinds of entanglement that can be generated and used expand rapidly. In a sense, with multipartite entanglement there is more than one type of entanglement, and one type of entanglement cannot be transformed into another type of entanglement just by the local parties performing operations locally. For the three parties, or tripartite entanglement, there are two types of states: the GHZ states and the W states.
This paper is focusing more on the GHZ state, which is a superposition of the three parties being in an entangled state like the 000 +111, whereas a typical Bell state can be 00 +11. We’ll focus on tripartite entanglement for now, but with multipartite entanglement there are more complicated forms of entanglement available, allowing more parties to be involved in entanglement-based applications and allowing different kinds of applications all together.
In bipartite entanglement, nodes are able to establish entanglement with a central node that uses entanglement swapping (mediated by a Bell state measurement), which is equivalent to teleporting entanglement to extend its range. Similarly, in multipartite entanglement, all parties establish entanglement with a central node. Instead of performing a Bell state measurement, it performs a GHZ state measurement which can always be done via some sort of interference between the photons (the qubits) followed by a measurement. Then it’s possible to distribute that state. In order to do this with three parties, for example, a kind of three party repeater, sometimes called a switch, is needed. This paper specifically explores two types of central node, and assumes there is access to quantum memories capable of storing the qubits until all parties are ready for measurement to happen - which will establish the multipartite entanglement.
The first type of central node is called a factory node, or an N-switch. Each end node establishes a Bell state with the factory node. Once all qubits are in place and in memory waiting, the node is able to then generate whatever state needs to be distributed - in this case, a GHZ state. Then the qubits are teleported to their respective nodes, i.e. Bell state measurements are performed and whatever state is generated in the central node is teleported to the end nodes. While the paper focuses on tripartite entanglement, in principle, this can be done with any number of multipartite entangled qubits. As long as the state of those qubits can be generated and then teleported to each end node via Bell state measurement, this is possible. The analysis in this research was restricted to just the GHZ states, but in principle it can be used for any end state.
The other type of central node this paper explores is called a 2-switch. This is a simpler central node than a factory node, and is essentially a repeater with memories in place that allows entanglement to be established independently by each node. In the 2-switch, the switch acts as a repeater for establishing a Bell state between 2 parties, like Alice and Bob. Then it establishes entanglement between a third party, for example Alice and Charlie. At that point, Alice has two qubits that are each entangled with a different party. A fusion operation can then be done to transform the state globally and to achieve a GHZ state - entangling all three parties.
In this paper, researchers simulate the performance of the factory node and the 2-switch. They look at the fidelity and the rate at which they can distribute GHZ states to the end nodes.
The primary takeaways from this analysis are that the factory node is more resilient to noise in the link that establishes elementary entanglement and to noise in the memory themselves in the central node. On the other hand, the 2-switch has its own advantages and disadvantages. The advantage of the 2-switch is that it is simpler. It only needs to be able to store two qubits and do a Bell state measurement. There are two potential downsides. A 2-switch requires a bit more work from the end nodes themselves: the end nodes must store more than one qubit and perform the fusion operation, which is a two qubit entanglement operation. Further, with a 2-switch, not all the multipartite entanglement states can be reached by this method.Beyond that, within the context of the analysis, a 2-switch performs better than the factory node if the Bell state measurements are probabilistic or noisy. If teleportation, perfect Bell state measurements, can be performed, then the factory node may be the better option. If Bell state measurements are a bit less than perfect, then the 2-switch is better at handling that noise.
The 2-switche is also better at scaling with the number of nodes: it can service a greater number of nodes, and also has a rate advantage in general over the factory node.
5. Quantum Internet Addressing
authored by Angela Sara Cacciapuoti, Jessica Illiano, Marcello Caleffi
https://arxiv.org/abs/2306.05982
From the abstract: “The design of the Quantum Internet protocol stack is at its infancy and early-stage conceptualization. And different heterogeneous proposals are currently available in the literature. The underlying assumption of the existing proposals is that they implicitly mimic classical Internet Protocol design principles: "A name indicates what we seek. An address indicates where it is. A route indicates how to get there''. Hence the network nodes are labeled with classical addresses, constituted by classical bits, and these labels aim at reflecting the node location within the network topology. In this paper, we argue that this twofold assumption of classical and location-aware addressing constitutes a restricting design option, which prevents to scale the quantumness to the network functionalities, beyond simple information encoding/decoding. On the contrary, by embracing quantumness within the node addresses, quantum principles and phenomena could be exploited for enabling a quantum native functioning of the entire communication network. This will unleash the ultimate vision and capabilities of the Quantum Internet.”
Of the research papers discussed here, this is the most speculative and most future-facing.
The authors are proposing we actually think about entanglement in quantum networking at a greater level of abstraction, where we can truly begin to take advantage of the weird way that quantum works - the different ways in which we can think about not just using networking for quantum, but rather using quantum for the process of networking itself.
In the context of quantum networking, we usually think about entanglement as being generated on request. For example, Alice and Bob request to teleport some states for secure communication. So then the process of generating entanglement is initiated, it’s used, then Alice and Bob teleport the state, and the request is fulfilled. In early development, this approach is necessary because entanglement is a difficult resource to distribute.
As quantum networks evolve to have more and more robust entanglement, that entanglement itself can be viewed as a form of connectivity: if Alice and Bob are entangled, then they can teleport a qubit from one side to the other regardless of physical connectivity. Therefore, entanglement itself is a form of quantum connectivity, and we can actually change where this connectivity is located using entanglement swapping. Entanglement can be viewed as adding to the topological neighborhood of your network instance. If two nodes are entangled, it doesn't matter how entanglement was established, they now have a connection and therefore, independent of whatever the actual physical quantum network looks like, there is an additional kind of connection in that network.
Imagining entanglement as a dynamically shifting topological augmentation of the overall network what this paper is all about. What the authors point out is that a classical network and a classical message on a network is only useful for where it's going, for its intended destination. Any node in between just passes that content on for the sole purpose of reaching the destination node. Entanglement, when it's established, is not like this. It is universally useful. If one of the nodes on the path from Alice to Bob suddenly needs entanglement a lot more, it’s possible to dynamically shift how the entanglement-resources in the network are distributed and prioritized..
We can imagine quantum networking in the future as there being a physical quantum network with a dedicated part meant to establish entanglement and store it preemptively. This preemptively stored entanglement can then be thought of as an overlay of that network. The researchers envision a physical network with dedicated supernodes, dedicated to performing a lot of entanglement between other supernodes. Once these entanglements are established, these super nodes are no longer just physical locations that we need to find to pass along a message, but are a piece of an entangled state, i.e. when you think of a network node address as a quantum system as opposed to a classical idea, a whole new range of capabilities are possible. This is the quantizing of the network. The path a quantum message actually takes along that network could itself behave quantumly for example: the photon that is carrying information can actually be in a superposition along multiple paths. Superpositions of paths, or of the temporal order of operations, and other quantum weirdness opens up a whole new world of communication and distributed processing, which we are just at the beginning of exploring.
As advancements in entanglement-based technology continue to unfold, understanding the nuances of these developments is crucial for anyone invested in the future of technology and communication. By staying informed and engaged with the latest research, we can better appreciate the potential and direction of Quantum Networking, ensuring we remain at the forefront of this revolutionary journey.
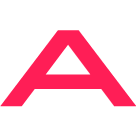